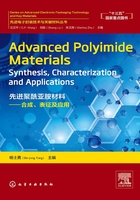
1.4 Structures and Properties of Polyimide Films
1.4.1 Advanced Polyimide Films
Advanced polyimide films have great combined properties, such as high mechanical strength and modulus, low thermal dimensional expansion, high adherence to metal and oxide material surfaces (copper, aluminum) and acceptable prices in market, etc., suitable for applications in manufacturing of high-density electronic packaging substrates. The common chemical structures of the advanced polyimide films were produced by the chemical imidization method derived from the aromatic dianhydrides (PMDA, or/and BPDA) and aromatic diamines (ODA, or/and PDA), etc. The representative successful commercial products in the current market include Kapton EN, Upilex S, Apical HP, etc.
The thermal and mechanical properties of advanced polyimide films can be adjusted by appropriately selecting the ratios of the aromatic dianhydrides such as PMDA and BPDA and diamines such as ODA and PDA in the formation of PAAs [33]. The PI-(BPDA/PDA) film has the highest modulus, the highest Tg, and the lowest CTE value among the polyimide films derived from BPDA, PDA, and ODA. These best properties are attributed to the rigid chemical structure of BPDA and PDA due to their nonflexible linkages in the molecular backbone structures. On the other hand, ODA has a flexible ether linkage due to the possibility of bending and rotation of the molecular chain. As the mole ratio of PDA/PDA+ODA in the copolyimide PI-(BPDA/PDA-ODA) films increases, the elastic modulus increases accordinglys from 3.8×10-6 to 7.8 GPa (Fig. 1.20) and Tg increases from 290 °C to 420 °C (Fig. 1.21), while CTE decreases from 36×10-6 to15×10-6 °C−1. It was found that even if a small proportion of ODA is incorporated with the polyimide backbone, the structure is disturbed and the obvious drop in Tg occurs, indicating that a simple additivity of component could cause obvious changes in Tg and that the effect of component would be sensible for rigidity.

FIGURE 1.20 Elastic modulus of the three-component copolyimide(BPDA/PDA-ODA)films.Open circles:experim- ental data;dashed line:estimated data.

FIGURE 1.21 Tg of the three-component copolyimide (BPDA/PDA-ODA)films.Open circles:experimental data;dashed line:estimated data.
In addition, the homopolyimide PI-(PMDA/PDA) film was expected to show the higher modulus and Tg than the homopolyimide PI-(BPDA/PDA) systems due to the greater rigidity of PMDA compared to BPDA. Unfortunately, the homopolyimide PI-(PMDA/PDA) film prepared is too brittle to measure the mechanical properties. Even the copolyimide PI-(PMDA/PDA-ODA (50/50)) film also showed a very low elongation (19%) and higher CTE (33×10-6 °C−1). The copolyimide films PI-(BPDA- PMDA/PDA) have large elongation at breakage, high modulus, as well as low CTE, when the mole ratio of BPDA/MPDA is greater than 50% (mol). On the other hand, the copolyimide films PI-(BPDA-PMDA/ODA) showed much higher CTEs and elongation at breakages than that of the corresponding PI-(BPDA-PMDA/ PDA) film.
Compared with the three-component copolyimide films derived from BPDA, MPDA and PDA, the four-component copolyimide films derived from BPDA-PMDA and PDA-ODA showed complex relationships between the thermal and mechanical properties and the polymer backbone structures. Only 10%—30% (mol) of ODA was allowed to incorporate in the copolyimide backbone for balancing of CTE and elongation at breakage of the films. High ODA loadings resulted in the CTE increasing significantly. The copolyimide films derived from BPDA-PMDA (50/50) and PDA-ODA showed almost linear relationships between the CTE and the PDA loadings (Fig. 1.22) and between the elastic modulus and the BPDA loadings (Fig. 1.23). The copolyimide film was prepared derived from BPDA-PMDA (50/50) and PDA- ODA (70/30), which showed the best combination of properties with CTE of 19×10-6 °C−1, elongation at breakage of 60%, modulus of 5.0 GPa, and Tg of 360 °C, successively.

FIGURE 1.22 CTE of the four-component copolyimide(BPDA-PMDA/PDA-ODA)films. Open circles:experimental data;dashed line:estimated data.

FIGURE 1.23 Elastic modulus of the four-component copolyimide(BPDA-PMDA/PDA-ODA(90/10))films.Open circles:experimental data;dashed line:estimated data.
Table 1.7 compares the properties of the three-component and four-component copolyimide films. A trade-off correction was clearly observed between the mechanical properties and the thermal properties, and the balanced properties of the polyimide films can be controlled, to some extent, by adjusting the polyimide backbone structures.
TABLE1.7 Properties of Three-and Four-Component Copolyimide Films

Because the apparent viscosity of PAA resin is mutually restricted by its solid concentration and/or polymer molecular weight, the PAA resin solutions derived from rigid aromatic dianhydrides and diamines always have too high solution viscosities for casting films on the support surface. Hence, PAA resins with relatively high concentrations (≥15%) and low solution viscosity in the range of 10 mPa·s-30×104 mPa·s at room temperature are desired for the manufacture of polyimide film with high modulus and low thermal expansion.
In order to determine the influence of PAA molecular weights on their solution-cast processing performance, a series of molecular-weight-controlled polyimide precursors (PI-PEPA) with designed calculated Mw were prepared from the reaction of BPDA and PDA using PEPA as a chain-extendable and end-capping reagent at concentrations of 20% in DMAc, as depicted in Fig. 1.24. The molecular weights were controlled by termination main-chains with the calculated molar fractions of PEPA to yield PAAs with calculated Mw of 5×103 g·mol−1to 50×103 g·mol−1. An analogous series of the unreactive phthalic-end-capped PAA resins (PAA-PA) with equal controlled Mw of 5×103 g·mol−1-50×103 g·mol−1 was also made for comparison.

FIGURE 1.24 Synthesis of molecular-weight controlled polyimides.
1.4.1.1 Film-forming Ability of Molecular-weight-controlled PAAs
The suitable apparent viscosity of PAA resin with high concentration is an important solution-cast processing parameter for the large-scale production of high-quality polyimide films. Fig. 1.25 shows the dependence of solution viscosity on the concentration for the molecular-weight-controlled PAAs with calculated Mw of 25×103 g·mol−1 and the molecular-weight-uncontrolled PAAs. A systematic rising trend in the apparent viscosities is observed with increasing concentration, and two regions with quite different slopes suggest critical concentrations (c* and c*′) at about 10% for PAAs (BPDA-PDA) without molecular weight limitation and 20% for molecular-weight-controlled PAAs (PAA-PEPA-25 and PAA-PA-25), respectively. It is noteworthy that the apparent viscosity of PAA resin varies as the fifth power of the concentration as the concentration exceeds 10%, and reaches over 9000 Pa·s with the concentration in excess of 20%.

FIGURE 1.25 Dependence of apparent viscosity on concentrations of the molecular-weight-controlled PAAs with calculated Mw of 25×103 g·mol−1 and the molecular-weight-uncontrolled PAAs.
In comparison, apparent viscosities of PAA-PEPA-25 and PAA-PA-25 are no more than 82 Pa·s with the concentration below 20%, after which an abrupt growth is noticed by a smaller exponential rate, proportional to the cube of PI precursor concentration. The observed huge disparity in the concentration dependence of solution viscosity indicates that molecular weight control is a promising pathway to readily adjust PAA resin to the desired practical film solutioncast processing conditions [3,4].
Fig. 1.26 describes the molecular weight dependence of apparent viscosity for molecular-weight- controlled PAA resins with concentration of 20% in DMAc at 25 °C. The apparent viscosities of both PAA-PEPA and PAA-PA increase gradually to 120 Pa·s when PAA calculated Mw≤30×103 g·mol−1, after which the seemingly exponential growths to 1160 Pa·s are displayed, rising in decreasing order: PAA-PA > PAA-PEPA. Obviously, the critical calculated Mw for molecular-weight-controlled PAAs imply that the overlapping of PAA main-chains and complexation-mediated solubilization between amide-acid chains and solvent become more prominent as the calculated Mw are over 30×103 g·mol−1. [5] The interactions and physical-type entanglements between polymer chains, primarily driven by hydrogen bonds and charge-transfer complexes (CTC), are more likely to play critical roles in concentrated solutions and govern their apparent viscosities, leading to the observed phenomenon that the molecular weights studied of molecular-weight-controlled precursors strongly affect the apparent viscosity behavior. Moreover, PAA-PEPA series might possess weaker internal friction upon shear deformation at the same controlled calculated Mw than PAA-PA ones. Therefore, introduction of PEPA end-capping into BPDA-PDA main-chain could be more effective to improve the solution-cast processability.

FIGURE 1.26 Molecular weight dependence of apparent viscosity for the initial molecular-weight-controlled PAA with concentration of 20%.
1.4.1.2 Mechanical Properties of Fully Cured Polyimide Films
The fully cured PI films were fabricated by classical two-step polymerization method in which the aforementioned molecular-weight-controlled PAA-PEPA resins with appropriate viscosities were thermally activated cyclized and successively stepwise-cured finally at 400 °C/1 h. For comparative purposes, the aforesaid PAA-PA controls end-capped with unreactive phthalic groups were thermally imidized up to 370 °C/1 h to yield typical linear PI films specified as PI-PA. All the films obtained were smooth, creasable, and yellow to orange in color.
Mechanical property comparatively recorded for PI-PEPA and PI-PA as a function of PAA calculated Mw is plotted in Fig. 1.27 and representatively summarized in Table 1.8. The tensile strength of PI-PEPA-5 to PI-PEPA-20 with PAA-PEPA calculated Mw≤ 20×103 g·mol−1, as illustrated in Fig. 1.27A, increases impressively by 38%, reaching 231.7 MPa. And then it appears to level off at 237.6 MPa when calculated Mw is above 20×103 g·mol−1. This suggests a critical calculated Mw of PAA-PEPA at around 20×103 g·mol−1, above which very small rises in the molecular weights do not affect the strength remarkably. In contrast, each film in PI-PA series has much lower strength at the identical calculated Mw value. For instance, PAA-PAs with calculated Mw < 15×103 g·mol−1 were not transformed to the free-standing PI films, because weak molecular interactions cannot offset internal stresses of shrinkage in the imidization process. But PAA-PEPA-5 was successfully converted to PI-PEPA-5 with the strength stabilized at 168.5 MPa, presumably by virtue of chain-extension effect occurring in the phenylethynyl moiety [34,35]. In Fig. 1.27B, the elasticity modulus of PI-PEPA decreases slightly from 7.7 to 7.3 GPa as the theoretical PAA-PEPA Mw grows to 20 × 103 g·mol−1, probably due to the reduced chain crosslinking densities; it becomes comparable for both PI versions with a further rise in PAA calculated Mn. The elongations at break (Fig. 1.27C) see a steady increase in the whole PAA calculated Mw of 382% and 325% for PI-PEPA and PI-PA, respectively. And PI-PEPA samples show generally higher elongations to failure; e.g., PI-PEPA-25 exhibits elongation of 8.5%, 39% higher than PI-PA-25 (6.1%).

FIGURE 1.27 Film mechanical property as a function of PAA calculated Mw(A)tensile strength;(B)elasticity modulus;(C)elongation at breakage.
TABLE1.8 Typical Mechanical Properties of the Fully Cured Polyimide Films Versus Linear Filmsa

The mechanical property variation is principally related to the molecular weight of PAA. The fully cured PI films obtained from reactive phenylethynyl-functionalized PAAs with calculated Mw>20×103 g·mol−1 surprisingly demonstrate much higher tensile strengths and elongations to deformation as compared to the linear polyimide controls with unreactive phthalic end-groups. Generally, the physical property is sensitive to the state of molecular interactions such as crosslinking density, the degree of crystallinity, and molecular stacking. Hence, the conspicuous enhancement in the tensile property of the fully cured PI film is assumed to be favored by the evolution in the aggregation structure effectuated by the thermal-curing reaction of phenylethynyl end-groups at elevated curing temperatures.
1.4.1.3 Effect of Thermal Curing on Aggregation State of Polyimide Films
The aggregation structure of polyimide film is intensely affected by the variables that control the thermal-curing process, e.g., temperature and time, which in turn would directly play a critical role in dictating mechanical and thermal properties [36,37]. It was expected that thermal transitions and concomitant morphology changes caused by the thermally activated, free-radical- predominant curing of phenylethynyl functional group under various processing conditions should be indicative of the curing behavior and mechanical response of PI films. PAA-PEPA-25 was selected to experience each curing procedure compared with PAA-PA-25.
Fig. 1.28 depicts the impact of thermal-curing processes on the crystallization behavior and morphological features of PI-PEPA-25 and PI-PA-25 films characterized by WAXD. Broad amorphous halos are visible for both series of films heated at lower temperatures (300 °C). And yet the relative intensities of three distinguishable diffraction peaks superimposed in the range of 18°-26° increase drastically, and each peak’s half-width decreases with rising in the final curing temperature from 370 °C to 400 °C, which reveals a rapid enhancement in the regularity of intramolecular arrangements in polyimide films [38]. Interestingly, the crystalline peaks in PI-PEPA-25 ultimately thermally processed at 450 °C transform to obtuse and less-structured patterns, indicating a reduction in chain ordering, while that in PI-PA-25 counterpart becomes sharper. The semicrystallized state disparity could be ascribed to the fact that the presence of the phenyl-ended moiety in the chain-extension structure marginally widens the extended molecular chains of layer structure in eclipsed crystals, thereby generating less-dense chain stacking and slight depression in the Tm [39,40].

FIGURE 1.28 XRD patterns of PI-PEPA-25 films(A)and PI-PA-25 films(B) cured at different temperatures,and the effect of final curing temperature on the ratio of crystalline region(C)
The effect of curing temperatures on the ratios of crystalline regions estimated from the diffractogram of the resulting PI film is compared in Fig. 1.28C. As the final heating temperature is ramped from 350 °C to 425 °C, the degrees of crystallinity in PI-PEPA-25 are 7.22%, 14.68%, 24.98%, and 27.26%, respectively, appearing to increase linearly. And the remarkable discrepancies of 2.17%, 6.95%, 3.89%, and 4.79% are observed as well, implying that the curing reaction occurring in the phenylethynyl moiety strengthens the ability to crystallize. According to Lambert et al. [41] the negligible proportion of the bulky crosslinks (cyclotrimer and polyene) significantly improves the rate of secondary nucleation in a way which causes the growth rate to increase drastically with decreasing crosslinking density, and the chain-extension moiety can be incorporated into the crystals. The cyclodimer as strong intermolecular bond localized at chain ends evolves gradually and constrains conformational freedom in the main-chain with elevated temperatures. It is reasonable to assume that the suppression of chain mobility due to the chain-extension chemistry is favorable to developing sparsely packed chains, inducing the ordered phase more easily than the rearrangement in intrinsically extended segments in the linear PI-PA-25 control [42].
1.4.1.4 Effect of Thermal Curing on Molecular Orientation and CTE of PI Films
The effects of curing procedures on molecular orientation and thermal dimension stability of polyimide films prepared from PAA-PEPA-25 and PAA-PA-25 are contrastively explored in Fig. 1.29. Both films with BPDA-PDA based backbone architectures show large persistence lengths in the chain direction and low chain flexibility, and the high birefringence (Δn) and low CTE consistent with a high level of in-plane chain orientation are normally manifested.
In Fig. 1.29A, the uncured polyimide film bearing the reactive phenylethynyl end-cap has lower birefringence and much higher CTE than the linear control with the unreactive phthalic end-group, declaring a great influence of phenylethynyl moiety on chain configuration by hindrance to rotation. The birefringence associated with the optical anisotropy increases by 33.7% for PI-PEPA-25, as compared to 15.9% for PI-PA-25 control, until the PAA films are ultimately thermally treated at 370 °C. In fact, the super-molecular structure is governed by the competition in orientation-relaxation in the polymer [43]. The rapid increase in the extent of molecular alignment parallel to the thermally cured film plane could be entailed not only by the fast upward trend in crystalline order as testified by WXRD, but also by the formation and retention of regular packing in molecular chains because of charge-transfer interactions over several consecutive repeat units in the amorphous regions [6]. At the same time, relaxation originated from random thermal motions could be effectively prevented by crystalline domain and short-range order region especially when the final curing temperature in CP1-CP3 is well below the Tg. But PI-PEPA-25 finally heated from 425 °C to 450 °C shows reduced birefringence and conversely PI-PA-25 counterpart exhibits slightly increased Δn. This tendency is similar to that in the degree of crystallinity, since the in-plane orientation engendered by short-range order is likely to be fairly countered by segmental relaxation above the Tg [42].

FIGURE 1.29 (A)Birefringence and (B) CTE of PI Films thermally treated at various final curing temperatures.
As expected in Fig. 1.29B, the CTE of the thermally cured material derived from PAA-PEPA-25 decreases dramatically from 8.5×10-6 to 5.1×10-6 °C−1, while that of PI-PA-25 is on the rise when the final curing temperature is set from 350 °C to 400 °C. This apparent disparity is associated with the different reasons for alterations in the birefringence and the CTE: the growth in Δn depends on the increase in a net rise in the preferential alignment of the imide rings in the in-plane direction; the increase in the CTE is contingent on the upward level of in-plane orientational relaxation. Nonetheless, the CTE data increase by around 18.4% for both PI films upon ultimately conditioning at 400 -450 °C.
1.4.1.5 Effect of Thermal Curing on Mechanical Properties of Polyimide Films
In order to further apprehend the thermal-curing effect of phenylethynyl moieties in the relatively high-molecular-weight PI film, the influence of the curing procedure on the mechanical properties of PI film is evaluated in Fig. 1.30. There is an optimal curing procedure (CP3) with the ultimate temperature as high as 400 °C for phenylethynyl-end-capped PAA resin (e.g., PAA-PEPA-25) transforming to the fully cured polyimide film (PI-PEPA-25). Tensile strength and elongation at break increase by 55% and 83% with advancement of the final curing temperature to 400 °C, reaching the highest figures (247.1 MPa and 8.6%). And then they drop substantially to 186.7 MPa and 3.4%, respectively, showing that the failure mode has turned to brittle fracture. A similar trend is visible for the linear PI-PA-25, and the largest strength and elongation of the material heating up to 370 °C are 25% and 26% lower than PI-PEPA-25. By increasing the curing parameters studied, the alterations in the crosslinking structures triggered by thermal-curing reaction, densification, and then thermo-oxidative degradation drives the elasticity modulus of both PI films to rise monotonously as anticipated.

FIGURE 1.30 Effect of curing temperature on mechanical property of PI films(A)tensile strength;(B)elasticity modulus;(C)elongation at breakage.
On the basis of the deduction from WXRD and thermomechanical analyses, the highly disordered crystals in intramolecular chains develop faster with evolution in chain-extension structures by the curing reaction of phenylethynyl moieties until the final curing temperature proceeds to the critical point (400 °C). The plastic deformation of aromatic PIs can be interpreted in terms of local order consisting of bundles of parallel-packed main-chains [7,8]. Consequently, relatively more microcrystals and short-range ordered domains with stronger molecular interactions could reinforce and toughen PI film. However, once the final curing step exceeds the critical point, the disorientation and degradation of molecular chains occur in the amorphous phase and crystalline area, holding the key to disrupting polymer chains and breaking ordered fractions.
Overall, the molecular-weight-controlled PAA resins end capped by reactive phenylethynyl groups with calculated Mw > 20×103 g·mol−1 exhibit moderate viscosities and high solid concentrations, showing enhanced wetting/spreading ability to form continuous PAA films. After thermal curing at 400 °C/1 h, the PAAs are successfully converted to the fully cured polyimide films which display better mechanical properties and thermal resistances than the high-molecular-weight, unreactive phthalic end-capped control, suitable for high-temperature microelectronic packaging applications. This property improvement may be put down to the thermal-curing effect of phenylethynyl end-groups on promoting ordering/packing of chain segments, resulting into an increment in the molecular interactions to reinforce and toughen PI films.
1.4.2 Low-CTE Polyimide Films
Aromatic polyimide films usually have much higher linear CTEs in the film plane direction ((40 - 80)×10-6 °C−1) than those of metal substrates (e.g., 17×10-6 °C−1 for copper foil). When polyimide films were formed on a metal substrate via thermal imidization after the solution casting of PAA solution, the polyimide/metal laminates undergo thermal stress arising from the CTE mismatch during the cooling process from cure temperature to room temperature. Consequently, serious problems are caused such as curling, cracking, and detaching of polyimide films. Considerable efforts have been made to attempt to decrease the film’s CTEs. Systematic researches on the structure-CTE relationship in various aromatic polyimide systems revealed that low-CTE polyimide films (<20×10-6 °C−1) have unexceptionally linear/stiff polyimide chain backbones. A typical low-CTE polyimide film is the Upilex S based on BPDA and PDA, which exhibits CTE values in the range of 5×10-6 to 20×10-6 °C−1 depending on the film thickness and processing conditions. The low CTE characteristics are closely related to a thermal imidization-induced in-plane chain orientation phenomenon.
Recently, demands in electronic applications have required polyimide films with both CTE and coefficient of humidity expansion (CHE), apart from the excellent mechanical properties. For PFC applications, adhesive-free polyimide film/Cu laminates (FFL, flexible copper clad laminates) are usually fabricated by direct coating of PAA solution onto copper foil. In this case, the CTEs of polyimide films must be precisely controlled to avoid the serious problems mentioned above. Another important requirement for polyimide films is the dimensional stability against water absorption or to decrease water absorption itself. In general, due to the highly polarizable imide groups in the backbone structures, polyimide films absorb water more easily in air than polyester films. For instance, commercially available Kapton H film (PI-(PMDA/ODA) has a moisture uptake of 2.5%-2.8%(w), much higher than polyester films (0.4%-0.6%). It is known that the lower water absorption characteristics of polyester films are attributed to the ester linkages in their backbone structures. Hence, polyimide films with ester-linkages in their backbone structures have been considered to be useful for reducing water absorption.
1.4.2.1 Ester-Bridged Low-CTE Polyimide Films
Hasegawa et al. have systematically investigated the structure-property relationship of polyimide films with ester-linkages in the polymer backbones (poly(ester-imide)s, PEsI) [44]. An ester-containing dianhydride monomer (Fig. 1.31), bis(trimellitic acid anhydride)phenyl ester (TAHQ), was used to react with various aromatic diamine monomers with stiff/linear structures, such as PDA, trans-1,4-cyclohexanediamine (CHDA), 2,2-bis(trifluoromethyl)benzidine (TFMB), 4-aminophenyl-4-aminophenyl -benzoate (APAB), and 4,4-daminobenzanilide (DABA) to give PEI precursors (PEsAA) solution, which was then cast on substrate to form PEsAA films and thermally imidized at high temperatures to give PEsI films.

FIGURE 1.31 Molecular structure of TAHQ.
Experimental results indicated that the PEsI films exhibited extremely low linear CTE values (3.2×10-6 °C-1 for PI-(TAHQ/PDA) and 3.3×10-6 °C−1 for PI-(TAHQ/APAB), respectively). The para-ester linkages behave as a rod-like segment, favorable for thermal-imidization-induced in-plane orientation. Copolymerization with flexible 4,4′-oxydianiline (ODA) made precise CTE matching possible between PEsI/copper substrate, with a significant improvement in film toughness at the same time. Hence, the introduction of ester linkages into the polyimide backbone has been considered an effective pathway to produce polyimide films suitable for use as PFC substrates. The polyimide films possess not only a copper-level low CTE but also high film toughness, high dimensional stability, and low water absorption.
TAHQ exhibits good polymerizability with aromatic diamines. The reduced viscosities (ηred) of the PEsAAs obtained by polycondensation of TAHQ and diamines in DMAc or NMP were measured in the range of 1.10-5.19 dL·g−1, indicating the high molecular weights of the PEsAAs. Hence, TAHQ has sufficiently high reactivity.
A series of TAHQ-derived homo-PEsI films, PI-(TAHQ-PDA), PI-(TAHQ-CHDA), PI-(TAHQ- TFMB), and PI-(TAHQ-ODA), were prepared. The combinations of three diamines (PDA, CHDA, and TFMB) with TAHQ are expected to give low CTE owing to their rigid/linear chain structures favorable for the in-plane orientation. And ODA was used as a typical flexible structure of diamine, expected to be effective as a comonomer for the improvement of film toughness. PI-(TAHQ-PDA) film is a high-quality, yellow-colored clear film. No cracks were observed on a 180 °C folding test, showing good flexibility. No distinct glass transition temperature up to 450 °C was observed, displaying an excellent dimensional stability. This may be attributed to suppressed molecular motions (internal rotation) in the amorphous regions and a semicrystalline morphology as mentioned later. PI-(TAHQ/PDA) film exhibited an extremely low CTE (3.2×10-6 °C−1), comparable to that of silicon wafer and a considerably high tensile modulus (E) of 8.86 GPa and strength (224 MPa). A high birefringence (Δn=0.219) was measured, corresponding to high in-plane orientation in the films. The elongation at breakage (Eb) representing toughness was not sufficiently high (Eb=5.4%) and the water absorption was 1.6%. In comparison, PI-(TAHQ/TFMB) also showed similar excellent properties such as no glass transition or high Tg behavior, high tensile modulus and strength, and low water absorption. However, the CTE was increased to 31.5×10-6 °C−1. In contrast, PI-(TAHQ/ODA) film showed common properties with the flexible-chain PI films, i.e., high CTE=51.2×10-6 °C−1 and low E=2.9 GPa. However, the Tg was unexpectedly high compared with other ODA-derived films. Fig. 1.32 depicts the backbone structures of three ODA-based films. PI-(TAHQ/ODA) film shows a Tg of 320 °C, much higher than that of the corresponding ether-linked PI-(HQDA/ODA) film (Tg=245 °C) and that of PI-(BPDA/ODA) film (Tg=245 °C) [30], respectively, indicating that the internal rotations are suppressed in the ester-linked polymer backbone structures. The PI-(TAHQ/ODA) film has very high toughness (Eb=67.2%) in contrast to PI-(TAHQ/PDA). Regarding water absorption properties, PI-(TAHQ/PDA) film showed obviously lower water absorption (1.6%) than the conventional Kapton H film (2.5%) due to the substitution of imide groups by ester groups in the polymer backbone structures. It is notable that the lower water absorption of the PI-(TAHQ/ODA) (0.6%) is comparable to that of fluorinated PI-(TAHQ/TFMB) film (0.7%). These results give a hint that copolymerization using flexible ODA can provide the balanced properties attaining low CTE, high Tg, low water absorption, and high toughness.

FIGURE 1.32 Molecular structures of ODA-based PI films.
Polyimide films with stiff main chains such as PI-(BPDA/PDA) and PI-(PMDA/ODA) tend to have complex morphologies in contrast to common semicrystalline polymers such as PET, which are composed of a simple crystalline/amorphous two-phase morphology. This is due to insufficient molecular mobility, and thereby the chain rearrangement for crystallization is disturbed. PI-(TAHQ/PDA) film displayed a much stronger and sharper pattern peaking at 2θ=21.6 degrees than PI-(BPDA/ODA) film, suggesting a semicrystalline structure. The crystallinity in the PI-(TAHQ/PDA) film probably contributes to the unclear Tg behavior observed in the E′ curve. The CTE may also be influenced by its crystalline morphology. It was concluded that CTE is primarily governed by the extent of in-plane orientation rather than crystallinity. Principally, crystallinity should also control the extent of water absorption (Table 1.9).
TABLE1.9 Properties of TAHQ-derived PEsl Films

In addition, an ester-containing aromatic diamine, APAB, was also used to react with the stiff/linear aromatic dianhydrides including PMDA, BPDA, and TAHQ to prepare the PEsI films. The reduced viscosities of the APAB-derived PEsI films were measured in the range of 1.09dL·g−1-2.81 dL·g−1, indicating a sufficiently high polymerization reactivity of APAB. The cast films were all highly flexible.
Table 1.10 summarizes the properties of APAB-derived PEsI films. It is noteworthy that both PI-(PMDA/APAB) and PI-(BPDA/APAB) films showed extremely low CTE values comparable with that of silicon wafer in accordance with considerably high birefringence. Thus, the results of the TAHQ- and APAB-derived PEsI films revealed that the para-aromatic ester linkages play a great role in the imidization-induced in-plane orientation, resulting in low CTE values. All of the APAB-derived PEsI films showed no appreciable glass transitions detected by DMA. High tensile modulus and high strength were also acquired in addition to high thermal stability.
TABLE1.10 Properties of APAB-derived PEsl Films

PI-(TAHQ/APAB) film exhibits similar properties to PI-(PMDA/APAB) and PI-(BPDA/APAB) films. The film toughness was somewhat improved by the combination of TAHQ and APAB. PI-(TAHQ/APAB) film also has a low water absorption. A sharp and strong reflection peaking at 22.0 degrees was observed in WAXD pattern, implying the presence of a semicrystalline morphology. As mentioned above, the chain linearity/rigidity is the most important factor for imidization-induced in-plane orientation (low CTE generation). This situation is seen in the comparison of the tensile modulus (E) and CTE between PI-(PMDA/4,4-ODA) film (E=3.0 GPa, CTE=41×10−6 °C−1) and PI-(PMDA/3,4-ODA) (E=5.0 GPa, CTE=33×10−6 °C−1) [38].
Fig. 1.33 compares the chain vectors (arrows) and possible conformations of several polyimide films. The chain segment of the former is largely bent at the ether linkage. The chain vector in the latter is also bent at the ether linkage but the linearity of the vector is somewhat recovered by taking a crank-shaft-like conformation. The higher modulus (higher degree of in-plane orientation) of PI-(PMDA/3,4-ODA) can be interpreted in terms of its possible conformation with a higher chain linearity. The para-aromatic ester unit can also be regarded as a similar crank-shaft-like linkage.

FIGURE 1.33 Comparison of the chain vectors (arrows)and possible conformations(A)PMDA/4,4'-ODA,(B)PMDA/3,4’-ODA, (C)TAHQ/PDA,and (D)PMDA/APAB.
In order to gain PEsI films with the target balanced properties, ODA was used to copolymerize with the stiff/linear aromatic dianhydrides and diamines, giving three coployimide films, including PI-(TAHQ/PDA-ODA (70/30)), PI-(PMDA/APAB-ODA (60/40), and PI-(TAHQ/APAB-ODA (70/30). Table 1.11 compares the properties of the three copolyimids films.
TABLE1.11 Properties of the Copolyimide Films

The PI-(TAHQ/PDA-ODA(70/30)) film shows good combined properties, including a very low CTE (11.7×10−6 °C−1), a relatively low water absorption (1.0%), and a high toughness (Eb=35.0%). In comparison, PI-(PMDA/APAB-ODA (60/40)) film also exhibits a very low CTE (11.9×10−6 °C−1) and sufficient toughness (Eb=25.5%), but a high water absorption (2.5%), probably due to the presence of the PMDA/ODA sequence. PI-(TAHQ/APAB-ODA(70/30)) film also shows a great combination of thermal, mechanical, and water-resistant properties, including a low water absorption (0.7%), a low CTE (14.8×10−6 °C−1), and a high toughness (Eb=35.8%). Obviously, the introduction of the flexible part into PI-(TAHQ/APAB) film is an effective pathway to accomplish the target combined properties.
Furthermore, the effect of substituents on the properties of the PEsI films with ester-linkages in the polymer backbones was systematically investigated [45]. The film properties of a series of PEsI films were evaluated for applications of high-temperature base film in FPC boards. Three ester-containing tetracarboxylic dianhydrides, including TAHQ, methyl-substituted TAHQ (M-TAHQ), and methoxy-substituted TAHQ (MeO-TAHQ), were employed to react with aromatic diamines such as PDA to give PEsAAs, which were then coated on glass and thermally imidized to PEsI films. The ester-containing monomers (TAHQ series and APAB series) were all highly reactive and led to PEsAAs possessing high inherent viscosities ranging from 1.09dL·g−1 to 9.33 dL·g−1. The incorporation of methyl- and methoxy-substituents into rigid TAHQ-based PEsI films caused no significant decrease in Tg, but allowed molecular motions above the Tgs. The substituents especially the methoxy group contributed to a significant decrease in water absorption without sacrificing other target properties. For practical FPC application, a flexible diamine (ODA) was copolymerized into the highly esterified rigid PEsI films. One of the PEsI copolymer films exhibited excellent combined properties: a low CTE (17.8×10−6 K−1) completely consistent with that of copper foil as a conductive layer, considerably low water absorption (0.47 %(w)), a high Tg (363 °C), and improved toughness (Eb>40%).
The ester-containing aromatic diamines, i.e., methyl substituted bis(4-aminophenyl) terephthalate (M-BPTP) and substituted 4-aminophenyl -4′-aminobenzoate (M-APAB) were used to prepare a series of the PEsI films (Fig. 1.34) [46].

FIGURE 1.34 Molecular structures of M-BPTP and M-APAB.
The PEsI films consisting of stiff/linear backbone structures and possessing lower CTE values than that of copper foil (17.7×10−6 °C−1) were obtained by a simple copolymerization approach using adequate amounts of flexible monomers to achieve the CTE matching in flexible copper-clad laminates (FCCLs) and drastic improvement of film toughness at the same time. Table 1.12 compares the combined properties of the PEsI films modified with flexible diamine. The incorporation of the ether-linkages by using 4,4′-ODA into PI-(TAHQ/BPTP) film improved the film toughness from Eb=4.5% to 12.7%, which was not so drastic an enhancement. The properties of PI-(TAHQ/M-BPTP) film were dramatically improved by copolymerization with 4,4′-ODA as indicated from a significantly enhanced Eb (50.7%) and a considerably reduced Wa (0.35%) as well as a controlled CTE (10.0×10−6 °C−1), although the concomitant decrease in the tensile modulus (from 7.74 GPa to 6.3 GPa) was not drastic.
TABLE1.12 Properties of PEsl Films Modified With Flexible Diamines

1.4.2.2 Fluorinated Low-CTE Polyimide Films
An effective method of reducing the moisture uptake of low thermal expandable polyimide films by attaching CF3 groups to the polymer backbone has been developed. A novel fluorinated ester-bridged aromatic diamine, bis(2-trifluoromethyl-4- aminophenyl)terephthalate (CF3-BPTP) was employed to prepare a series of fluorinated ester-bridged polyimide films with controlled ester-bridged segments and fluorine contents in the polymer backbone (Fig. 1.35). The PI films were prepared by copolymerization of BPDA as aromatic dianhydride monomer and the aromatic diamine monomer mixture consisting of PDA and different amounts of CF3-BPTP. Experimental results indicated that the film’s water uptakes (Wa) were reduced with increasing of the CF3 groups loadings in the ester-bridged polyimide backbones while keeping the films with low enough CTEs. By controlling CF3 group loadings, polyimide films with desirable combinations of thermal, mechanical, and dielectric properties for application in high-density and thinner FPCs have been obtained. Thus, polyimide films with CTE of ≤20×10−6 °C−1 at 50-200 °C, glass transition temperature (Tg) of ≥310 °C, Young’s modulus of ≥6.0 GPa, Wa of ≤1.2%, dielectric constant (ε) of 3.4 have been obtained. The two-layer flexible copper clad laminate (2L-FCCL) prepared by coating the polyimide precursor resin solution on the surface of copper foil followed by being thermally imidized at elevated temperature did not cause apparent curling due to their closed CTE values.

FIGURE 1.35 Synthesis of the fluorinated ester-bridged polyimide films.
1.4.2.2.1 Film Forming Ability
The effect of the ester-bridged diamine concentration on the PAA solution viscosities is shown in Fig. 1.36. The PAA-(BPDA/PDA) solution has very high viscosity, resulting in difficulty in casting films. As the fluorinated ester-bridged aromatic diamine (CF3-BPTP) is inserted in the polymer backbone structure of PAA-(BPDA/PDA) resin, the solution viscosity can be decreased significantly. As the mole ratio of CF3-BPTP/PDA was increased from 5% to 30%, the produced PAA resin solution viscosity decreased from 38.18 Pa·s to 2.17 Pa·s. In comparison, the unfluorinated esterbridged aromatic diamine (BPTP) only yielded the PAA resin solution viscosity descended from 86.70 Pa·s to 41.52 Pa·s under the same conditions, demonstrating that the fluorinated ester-bridged diamine was more effective than the corresponding fluorine-free one in reducing of the PAA resin solution viscosity, thus improving the film-casting property. This phenomenon might be interpreted by the presence of bulky CF3 substituent in the polyimide backbone, which inhibited the chain packing and weakened the intermolecular interactions of the rigid polymer backbones.

FIGURE 1.36 Dependence of PAA resin absolute viscosity on the ester-bridged diamine concentrations.
1.4.2.2.2 Film Mechanical Properties
The effect of the ester-bridged segment concent- rations in polyimide on film mechanical properties was shown in Fig. 1.37. The ester-bridged polyimide films showed higher Young’s modulus in the range of 6.0 GPa—6.9 GPa, comparable to that of the commercial PI-(BPDA/PDA) film (Upilex S, 6.0 GPa) at the same film thickness and treatment procedure. This could be ascribed to the following two reasons: (1) the polyimide backbone composed of a para-linked aromatic ester-bridged segment and a rigid BPDA-PDA skeleton has a high level of in-plane orientation; (2) the strong dipole-dipole interaction between the imide carbonyl groups and ester linkages in the polymer backbones exhibited strong inter-molecular interactions, resulting in a physical cross-linking effect in the polyimide morphology. Besides, with increasing loading of the ester-bridged segments in polymer backbone, the film’s modulus gradually reduced from 6.9 GPa (5 %(mol) CF3-BPTP) to 6.7 GPa (10 %(mol) CF3-BPTP), and to 5.9 GPa (30 %(mol) CF3-BPTP). Moreover, the tensile strengths of the ester-bridged polyimide films declined linearly from 185 MPa to 140 MPa with an increase in the concentration of the ester-bridged segments in polyimide backbone.

FIGURE 1.37 Effect of ester-bridged segment loading on polyimide film’s tensile modulus(A)and tensile strength(B).
In contrast, PEI-II series films derived from BPTP exhibited higher Young’s modulus and higher tensile strengths than PFEI-I series films at the same concentration of ester-bridged segments, indicating that the insertion of CF3 groups in the ester-bridged polyimide backbone resulted in the decline of both film’s modulus and strength probably due to its weaker concentration reactivity.
1.4.2.2.3 Water Uptake
The water uptake of aromatic polyimide film for high-density FPC application is a key issue. The lower the water uptake, the lower the humidity expansion of FPC. Fig. 1.38 shows the dependence of the ester-bridged segment loading in the polyimide backbone on the film water uptakes. The PFEI-I series films exhibited water uptakes decreased from 2.6% for PFEI-Ia (5%(mol) of CF3-BPTP) to 0.7% for PFEI-Id (30%(mol) of CF3-BPTP) with increasing of the ester-bridged segment loadings. This could be interpreted by the polymer structural factors: one was the decreased imide contents in the ester-bridged polyimides; another reason was the existence of the hydrophobic trifluoromethyl substituent in the polyimide backbone, which reduced the surface energy and intermolecular cohesive force, resulting in inhibition of the moisture adsorption. Moreover, the fluorinated ester-bridged polyimide films (PFEI series films) showed lower water uptakes than the corresponding fluorine-free ones (PEI-II series films).

FIGURE 1.38 Effect of ester-bridged segment loadings on polyimide film’s water uptakes(A)and surface energy(B).
1.4.2.2.4 Thermal Dimension Stabilities
The in-plane CTE of the fluorinated ester-bridged polyimide films were measured by TMA analysis, as shown in Fig. 1.39. The polyimide films showed small dimensional increases until the scanned temperature reached to the glass transition temperature, then the dimensional increases changed obviously (Fig. 1.39A). The CTE values increased linearly with the increasing of the ester-bridged segment loadings in the polyimide backbones (Fig. 1.39B). The PFEI-I0 had the lowest CTE of 3.3×10-6 °C−1 due to its most linear/rigid backbone structure, compared to 8.3×10-6 °C−1 of PFEI-Ia (5%(mol) of CF3-BPTP), and 18.3×10-6 °C−1 of PFEI-Id (30%(mol) of CF3-BPTP), respectively, probably attributed to the big free volume and looser chain packing induced by CF3 groups. On the other hand, the incorporation of fluorine-free BPTP did not cause drastic dimensional increase like the fluorinated CF3-BPTP, whose CTE values varied from 3.7×10-6 to 6.3×10-6 °C−1 with increasing of the ester-bridged segments. Hence, the rod-like para-aromatic ester-bridged segment behaved like the rigid poly(BPDA/PDA) skeleton, which was also an indispensable structure factor for the in-plane orientation, resulting in lower thermal expansion. Meanwhile, although appropriate insertion of CF3 substituent into polyimide backbone led to a slight increase in CTE value, it was beneficial for adjusting the CTE value precisely close to that of copper foil for FPC application. In the fluorinated ester-bridged polyimide films, PFEI-Id film possessed much closer CTE (18.3×10-6 °C−1) to that of copper foil (17.8×10-6 °C−1) and low water uptake of 0.7%(w), thus making it a desirable candidate for FPC’s substrate film.

FIGURE 1.39 Dimensional stabilities of the PFEI-I series films(A)and the dependence of CTE values on the ester-bridged segment loadings(B).
1.4.3 Transparent Polyimide Films
In recent years, aromatic polyimide films have been widely used in microelectronics and aerospace industries, however the colors from yellow to brown have limited their applications in optical and display devices. The coloration of the common aromatic polyimide films is primarily attributed to the intermolecular CTC formed due to the molecular aggregation between polymer chains in the solid states. With the rapid development of optoelectronic engineering, optical films with high thermal resistance are highly desired due to the increasing demands for high reliability, high integration, and high signal transmission speed in optoelectronic devices [47,48]. For instance, in the fabrication of flexible active matrix organic light emitting display devices (AMOLEDs), the processing temperature on the flexible polymer film substrates might be higher than 300 °C [49-51]. Most of the common polymer optical films, such as polyethylene terephthalate (PET) or polyethylene naphthalate (PEN) lose their optical and mechanical properties at such a high processing temperature. Thus, colorless and optically transparent polymer optical films with high-temperature resistance have attracted a great deal of attention from both the academic and engineering fields. This driving force has greatly promoted the development of optical polymer films with outstanding thermal stability.
Generally, optical polymer films can be divided into three types according to their servicing temperatures or glass transition temperatures (Tg), including conventional optical films (Tg < 100 °C), common high-temperature optical films (100 °C≤ Tg < 200 °C), and high temperature optical films (Tg ≥ 200 °C), as shown in Fig. 1.40. The typical chemical structures for the optical polymer films and their Tg values are illustrated in Fig. 1.41. Although conventional polymer optical films possess excellent optical transparency, the lower Tg (PET, Tg=78 °C, PEN, Tg=123 °C) limit their practical applications in advanced optoelectronic industries. Hence, high-temperature polymers such as polyamideimide (PAI), polyetherimide (PEI), and polyimide (PI), with high optical transparency have been considered as one of the hot topics in the advanced polymer optical film field in recent years.

FIGURE 1.40 Classification of optical polymer films.

FIGURE 1.41 Typical chemical structures and Tgs of the optical polymer films.
For development of colorless transparent polyimide (CTPI) films, one of the most challenging issues is to balance the thermal properties, the optical transparent properties, and the mechanical properties. Fig. 1.42 shows the molecular structure design for CTPI films, including favorable designs and unfavorable ones. The favorable designs that could improve both the high-temperature stability and optical transparency include introduction of alicyclic substituents, such as cyclobutane, cyclohexane, cardo groups (diphenylcyclohexane, adamantane, etc.); highly electronegative groups, such as trifluoromethyl (—CF3); and asymmetrical or twisted rigid substituents, such as asymmetrically substituted biphenyl or diphenylether moieties. These functional groups or structure segments have been widely adopted to develop novel CTPI films, endowing CTPI films with both excellent thermal stability (Tg ≥300 °C) and good optical transmittance (>85% in the visible light region). Simultaneously, these substituents or structure segments could prohibit the formation of CTC induced by the electron-donating and electron- withdrawing moieties in CTPI films.

FIGURE 1.42 Molecular structure design for colorless transparent polyimide films.
Monomer design and synthesis is a key issue in the preparation of CTPI films because most of the properties of CTPI films, including optical transparency, mechanical strength, CTE, etc., are dependent on the molecular structures of the starting monomers, including dianhydrides and diamines. Currently, the development of CTPI films has been hampered by the high cost of the limited commercially available monomers. Table 1.13 summarizes some typical commercially available monomers for CTPI films [52-60]. For these monomers, including dianhydrides and diamines, the alicyclic moiety or highly electronegative —CF3 or bulky —SO2— moieties in their structures are beneficial to reducing or inhabiting the intra- and/or intermolecular CTC formation in the derived CTPI films, thus improving their optical transparency. Meanwhile, the high chemical bond energies and bulky molecular volumes for these groups endow the derived CTPI films with good thermal stability. It was found that the dianhydride monomers have more effects on the properties of CTPI films than the diamines. Up until now, fluorine-containing or alicyclic dianhydrides have been intensively investigated to develop novel CTPI films. However, the high cost and synthetic difficulties have seriously hindered the rapid development and commercialization of CTPI films.
TABLE1.13 Typical Monomers for CTPI Synthesis

TABLE1.13 (Continued)

The reactivity and purity of monomers also have obvious influence on the physical and chemical properties of CTPI resins, including molecular weights and their distribution, inherent viscosities, solubility in organic solvents, appearance, color, and so on. These features have great effects on the combined properties of the final optical polymer films, including their color, optical transparency, mechanical strength, thermal stability, and dielectric properties. Besides, the film-forming technologies including casting procedure, the uniaxial or biaxial stretching process, high-temperature curing program, and even the final winding and rewinding process also have apparent influence on the combined properties of CTPI films. Hence, the manufacture of CTPI films is a multidisciplinary technology.
CTPI resin synthesis is another key feature in the development of high-quality CTPI films. Polycondensation is the stepwise reactions between bifunctional or polyfunctional components with the liberation of small molecules, such as water, alcohol, hydrogen halide, etc. Generally, the polycondensation reaction is carried out in fully dried polar solvents with high boiling points, such as dimethyl sulfoxide (DMSO), DMF, DMAc, NMP, g-butyrrolactone (GBL), and meta-cresol in order to achieve homogeneous reaction and obtain high-molecular-weight CTPI resins. In some cases, molecular weights of the CTPI resins have to be controlled in order to achieve good processability. A stoichiometric imbalance in the monomers (excess of one of the reactants over another) or addition of mono-functional reagent is usually used to control the molecular weight of the obtained polymers.
The synthesis of CTPI resins can usually be performed via two procedures, including a two-step synthesis via PAA precursor, followed by imidization reaction either at elevated temperatures up to 300 °C or by chemical dehydration reaction with acetic anhydride under the catalysis of pyridine or other organic alkalis (Fig. 1.43A); and a one-step high-temperature polycondensation procedure to produce polyimide directly (Fig. 1.43B). The first reaction consists of the polymerization of an aromatic diamine and a dianhydride at room temperature in a polar aprotic solvent (DMAc or NMP). The resulting PAA is soluble in the reaction medium. Then, the viscous PAA solution is cast into a thin layer on glass or stainless steel substrates and heated from room temperature to high temperatures up to 300 °C in inert atmosphere, resulting in the evaporation of solvents and ring closure with intramolecular elimination of water to facilitate the imidization reaction [61]. The obtained CTPI resins are usually not soluble in organic solvents. Alternatively, if the final CTPI resins are soluble in organic solvents, the PAA precursors can be imidized via a chemical dehydration procedure [62,63]. Similarly, organo-soluble polyimide resins can also be synthesized via a high-temperature polycondensation procedure [64]. In this procedure, as shown in Fig. 1.43B, the PAA precursor is not separated from the reaction medium, which is dehydrated in situ to form the CTPI resins. The solid preimidized CTPI resins are usually soluble in polar solvents and their solution can be cast into films at a relatively low temperature (evaporation of solvents). This is quite beneficial for producing colorless films.

FIGURE 1.43 Synthesis of transparent polyimide resins.
Overall, CTPI resins can be synthesized via various polycondensation procedures. Several critical factors, including the reactivity, purity, and stoichiometry of the starting monomers, and polymerization temperature and time will determine the final physical (molecular weight and its distribution, density, etc.) and chemical (solubility, solvent resistance, etc.) characteristics of the resulting CTPI resins. These features will directly exert an influence on the properties of the final CTPI films, such as optical, mechanical, thermal, and dielectric properties.
The fluorinated polyimides derived from 6FDA (2,2-bis(3,4-dicarboxyphenyl) hexafluoropropa- nedianhydride) and aromatic diamines are well known to be very transparent [65-69]. And the polyimides derived from an alicyclic diamine, DCHM (4,4′-diaminodicyclohexylmethane) and aromatic dianhydrides, also showed good transparency and thermal stability (Fig. 1.44). Hence, semialicycli polyimides have been investigated systematically to seek the colorless transparent polyimide films.

FIGURE 1.44 The chemical structures of the tran- sparent polyimide films.
The ultraviolet-visible absorption spectra and fluorescence spectra of PI (6FDA/DCHM) and PI (6FDA/PDA) films were investigated, as well as their thermal properties [70]. Both films showed high transparency in the visible region (Fig. 1.45), there are no absorption bands above 370 nm. The bulky group —C(CF3)2— prevents molecular packing in the polyimide films, resulting in weak intermolecular interaction between the diamine moiety as an electronic donor, and a di-imide moiety as an electronic acceptor. It is noteworthy that the absorption spectrum of PI (6FDA/DCHM) film exhibits a very small platform region from 260nm to 300 nm, and PI (6FDA/PDA) film shows a shoulder absorption at 260 nm, similar to that of Kapton H films. This is attributed to the intramolecular charge transfer. The very weak intramolecular charge transfer of PI (6FDA/DCHM) film, compared with PI (6FDA/PDA), can be explained by the weak electron-donating property of DCHM [69]. The absorption intensity for PI (6FDA/DCHM) above 300 nm is weaker than that for PI (6FDA/PDA), implying that intermolecular charge transfer in PI (6FDA/DCHM) is very weak even if it was formed, compared with that for PI (6FDA/PDA). It can be concluded that the introduction of 6FDA into polyimide chains would weaken the intermolecular charge transfer due to the steric hindrance [48]. On the other hand, the weak electron-donating property of DCHM would reduce not only intermolecular charge transfer but also intramolecular charge transfer. Thus, by introducing 6FDA and DCHM into polyimide chain, a very transparent polyimide film was obtained. Both films have Tgs of about 250 °C, indicating that thermal stability of polyimides was not reduced by introducing the alicyclic groups.

FIGURE 1.45 The UV absorption spectra of PI-(6FDA/ DCHM)(A)and PI-(6FDA/PDA)(B)thin film of 0.6μm.
A series of transparent polyimides were prepared derived from 2,3,5-tricarboxycyclopent- ylaceticacid dianhydride (TCAAH) with different aromatic diamines, including an aromatic diamine, 4,4′-diaminodiphenylmethane (DPM), a fluorinated diamine, 2,2-bis(4-diaminodiphenyl)hexafluoropropane (6FdA), and an alicyclic diamine, DCHM (Fig. 1.46). All of the polyimide films showed high transparency in the visible region, no absorption tail was found above 330 nm (Fig. 1.47). The absorption shoulders at 248 nm of PI (TCAAH/DPM) and PI (TCAAH/6FdA) are due to the absorption of phenyl groups in the diamine moieties. The Tgs of these polyimides were measured in the region of 251 °C-336 °C, and the decomposition temperatures were measured in the region of 427 °C-457 °C.

FIGURE 1.46 Chemical structures of transparent polyimides.

FIGURE 1.47 UV absorption spectra of transparent polyimide films(0.3 µm) (1)PI-(TCAAH/DCHM); (2)PI-(TCAAH/6FdA); (3)PI-(TCAAH/DPM).
A series of transparent polyimide films were prepared derived from 1,2,3,4-cyclobutanetetracarboxylic dianhydride (CBDA) with different diamines, including an aromatic diamine (MDA), a fluorinated diamine (6FdA), and an alicyclic diamine (DCHM) (Fig. 1.48). All of the films showed high transparency in the visible region, i.e., no absorption tail was found above 375 nm (Fig. 1.49). In particular, PI (CBDA/DCHM) film showed the highest transparent property with almost no absorption above 310 nm. Absorption shoulders at 235 nm of PI (CBDA/DPM) and PI (CBDA/6FdA) are due to the absorption of phenylene groups in the diamine moieties. The glass transition temperatures of the polyimides were measured at 277 °C-360 °C and the decomposition temperatures at around 450 °C under deaerated conditions.

FIGURE 1.48 Chemical structures of the transparent polyimides.

FIGURE 1.49 UV absorption spectra of the CTPI films(ca.0.3 µm).
Ha et al. have prepared a series of novel CTPI films, including fully aliphthatic polyimides either from adamantane-based diamines [71-73] or from an aliphatic dianhydride [74], highly transparent and hydrophobic fluorinated polyimides derived from perfluorodecylthio substituted diamine monomers [75], colorless PIs with chlorine side groups [76], fluorine-containing colorless PI-montmorillonites nanocomposite films [77], colorless PI-organoclay nanocomposite films [78], highly optically transparent PIs containing carbazole moieties [79], polynorbornene-chlorinated PI copolymers films [80], and optically transparent PI/graphene nanocomposite films [81]. Various applications for the developed colorless PI films as flexible substrates for organic electroluminescent devices [82] and as hole-transporting layers for hybrid organic LEDs [83] have also been investigated.
A highly transparent and colorless semiaromatic polyimide film has been prepared derived from alicyclic dianhydride and aromatic diamine at ICCAS (Fig. 1.50). A novel meta-substituted aromatic diamine containing trifluoromethyl and sulfonyl groups in the backbone, 2,2′-bis[4-(3-amino- 5-trifluoromethylphenoxy) phenyl]sulfone (m-6FBAPS), was synthesized, which was then used to react with 1,2,4,5-cyclohexanetetracarboxylic dianhydride (CHDA) to yield a semiaromatic polyimide film. In comparison, a series of other semiaromatic polyimides were also prepared from CHDA and various aromatic diamines.

FIGURE 1.50 Synthesis of semiaromatic polyimides.
The optical transparency of the semiaromatic polyimide films was evaluated by their physical appearance, UV-vis spectra and color intensities. These films were transparent and essentially colorless even as the film thickness was as high as 70 mm, being significantly different from the deep-yellow or brown color of traditional aromatic polyimide films (Fig. 1.51). The improved optical transparency is attributed to the effect of the alicyclic moieties in the polymer structure. The optical transparency obtained from the UV-vis spectra, including cutoff wavelength (absorption edge) and transmittance at different wavelength, are summarized in Table 1.14. All of the semiaromatic polyimide films showed excellent optical transparency with UV cutoff wavelengths of less than 314 nm and transmittance at 450 nm of higher than 91%. In comparison, the typical aromatic polyimide film derived from PMDA and 4,4′-ODA (ref-PIb) showed a deep-yellow color with transmittance at 450 nm of 2%.

FIGURE 1.51 UV-vis spectra of semiaromatic polyimide films(film thickness:25 mm). The insert is the photograph of ref-PIb film.
TABLE1.14 Optical Transparency of Semiaromatic Polyimide Films

The extremely transparent and entirely colorless films were obtained from the polymers incorporated with bulky electron-withdrawing trifluoromethyl- and sulfonyl- groups in diamine moieties, such as PI-2, PI-5, and PI-6, in which PI-6 showed the best transparency with T400 of 90% and T450, of 94%, respectively. Their excellent optical properties are attributed to the distorted molecular conformation combined with the weakened electron-accepting and electron-donating properties of dianhydride and diamines, which significantly restrained the formation of inter-/intra-molecular charge transfer interactions.
Furthermore, the method of preparing transparent and conductive indium tin oxide (ITO)/polyimide films by high-temperature radio-frequency magnetron sputtering has been developed at ICCAS. A highly transparent and thermally stable polyimide substrate (Fig. 1.52) was prepared and used for the fabrication of ITO/PI films via radio-frequency magnetron sputtering at an elevated substrate temperature. The effect of the deposition conditions, i.e., the oxygen flow rate, substrate temperature, sputtering power, and working pressure, on the optical and electrical properties of the ITO/PI films were investigated from the microstructural aspects. The results indicate that the optical and electrical properties of ITO were sensitive to the oxygen. Moreover, it was beneficial to the improvement of the ITO conductivity through the adoption of a high substrate temperature and sputtering power and a low working pressure in the deposition process. A two-step deposition method was developed in which a thick bulk ITO layer was overlapped by deposition on a thin seed ITO layer with a dense surface to prepare the highly transparent and conductive ITO/PI films.

FIGURE 1.52 Chemical structure of the transparent polyimide film.
The optical properties of the ITO-m/PI and ITO-b/PI films were evaluated by their physical appearances, transmittance spectra, and color intensities. Fig. 1.53 shows the film transmittance spectra and photographs. Both films were highly transparent and entirely colorless, showing average transmittances in the range 400nm-800 nm over 81% and values of b* less than 3 (Table 1.15). ITO-b/PI exhibited a very low resistivity of 9.03×10−4 Ω·cm, two orders of magnitude lower than that of ITO-m/PI. The difference in the conductivity could be explained by the surface morphology perspective. The ITO/PI film after annealing at 240 °C gave a transmittance of 83% and a sheet resistance of 19.7 Ω·m-2.

FIGURE 1.53 Transmittance spectra of the ITO/PI films.The insets show the photographs of (A)ITO-m/PI and(B)ITO-b/PI films.
TABLE1.15 Optical and Electrical Properties of the As-Deposited ITO-m/PI and/To-/PI Films and Annealed ITO-m/PI Film

Mitsubishi Gas Chemical Company reported a method for producing colorless and transparent PI films by a solution casting procedure. The bi-axially stretched colorless PI films exhibit excellent optical transparency, heat resistance, and reduced dimensional changes [84].
The films were produced with the soluble CTPI resin as the starting materials, which were derived from CBDA and aromatic diamines by a one-step high- temperature polycondensation route. The CTPI film was bi-axially stretched in the machine direction by 1.01 times and in the transverse direction by 1.03 times at 250 °C for 11 min under a stream of nitrogen. Then, the CTPI film was dried by blowing nitrogen containing 1000×10-6oxygen at a flow rate of 3.3 m·s−1 at 280 °C for 45 min. The obtained CTPI film had a thickness of 200 µm, a total light transmittance of 89.8%, a yellow index of 1.9, and a haze of 0.74%. The solvent residual ratio in the film was 0.5% by weight. Due to their unique optical and thermal properties, the colorless CPPI films might find extensive applications in optoelectronic applications, such as transparent conductive film, transparent substrates for flexible display, flexible solar cells, and flexible printing circuit board (FPCB). Similar procedures were also reported [85].
Additionally, DuPont Company [86] and Kolon Industries [87] have reported the methods of low-color polyimide films derived from the copolymers of fluoro-containing dianhydride, 2,2-bis(3,4-dicarboxyphenyl)-hexafluoropropane dianhydride (6FDA), BPDA, and fluoro-containing diamine, 2,2′-bis(trifluoro-methyl) benzidine (TFMB). The copolymers were prepared via PAA precursors, followed by chemical imidization of the PAAs to afford the gel-like PAA films or soluble CTPI resins. Then, the CTPI films were produced from these intermediates at high temperatures up to 300 °C. Flexible and tough CTPI films with low color and high transparency were obtained.
NASA (National Aeronautics and Space Administration, USA) Langley research center have investigated the molecularly oriented transparent polyimide films for space applications [88]. In large space structures with designed lifetimes of 10-30 years, there exists a need for high temperature (200 °C-300 °C) stable, flexible polymer films that have high optical transparency in the visible light region. For this purpose, a colorless and transparent polyimide film, LaRC-CP1, derived from 6FDA and fluoro-containing diamine, 2,2-bis[4-(4-aminophenoxy)phenyl] hexafluoropropane (BDAF), has been developed by NASA. This film is prepared from soluble polyimide resin. The LaRC-CP1 film was mono-axially stretched by 1.5, 1.75 and 2 times the original length of the film. The tensile properties of the film increased with increased stretching ratio. The tensile strength of 2.0× stretched film increased from 93.0MPa to 145.4 MPa after stretching treatment; and the elongations increased from 16% to 65%. After stretching treatment, the dimensional stability, stiffness, elongation, and strength of the films were greatly enhanced, which are crucial for the applications in space environments.
1.4.4 Atomic Oxygen-resistant Polyimide Films
Atomic oxygen (AO) and vacuum ultraviolet (VUV) can create hazardous conditions to degrade polymer materials used in the exterior surfaces of a spacecraft in low Earth orbit (LEO). Usually, the exterior surfaces of spacecraft are covered with multilayer thermal insulation (MLI), in which the outer surface of MLI consists of bare polyimide films, such as Kapton H, Upilex R, etc. The AO- and VUV-induced erosion of these polymer films always results in the deterioration of MLI properties. In order to protect polyimide films from AO bombardment, optically transparent inorganic thin films have been used as overlay protective coatings. The inorganic thin films used includes ITO, tin dioxide (SnO2), Al2O3, silicon dioxide(SiO2) or silicon dioxide mixed with PTFE Teflon, etc. [89]. However, there are three major problems in the current hard coating technology. One is pinholes in the protective coatings produced in the coating process. It was found that even small pinholes can cause serious undercutting in the polymer layers [90]. Hence, the number of pinholes or defects in the inorganic coating must be controlled to a very low level, enabling the polymer films to be survived. This is a big technological challenge for current thin-film processes. Another problem is the cracks produced at areas where cutting, punching, or a sharp bend is performed on the inorganic coated polymer films. The cracks in size are larger than pinholes, which are usually the major sources for MLI damage. The last problem is collision with space debris or micrometeoroids in orbit, which may result in openings in the inorganic coatings where AO can react with the polymer underneath. A hard coating with micrometer thickness can be easily penetrated by these high-energy particles.
The protective inorganic coatings are always used in the actual space systems such as the International Space Station; some of the coatings are quite durable when used properly but there are still some problems that occur due to their lack of self-healing at defect sites. Hence, protective coatings with self-healing capability is desired for the space crafts in LEO.
Kapton polyimide film is extensively used in solar arrays, spacecraft thermal blankets, and space inflatable structures. Upon exposure to AO in LEO, the film is severely eroded. An effective approach to prevent this erosion is to incorporate polydedral oligomeric silsesquioxane (POSS) into the polyimide backbone structures by copolymerizing the monomer mixture of POSS-containing diamine and aromatic diamine with aromatic dianhydride in a polar solvent to give high-molecular-weight PAA solution, which is then cast on a support surface to give a PAA film [91]. After removal of some part of the solvent by evaporation and partial chemical or thermal imidization, a self-standing gel film is peeled off from the support surface, followed by imidization to produce a POSS-Kapton polyimide film (Fig. 1.54). The POSS is a unique family of nanoscale inorganic/organic hybrid cage-like structures that contain silicon/oxygen framework (RSiO1.5), where R is a hydrocarbon group used to tailor compatibility with the polymer and chemical stability. Thus, a series of POSS-Kapton films with different POSS-diamine monomer loadings of 0%, 5%, 10%, 20%, and 30%, corresponding to Si8O11 cage loadings of 0%, 1.75%, 3.5%, 7.0%, and 8.8%, were prepared, including main-chain (MC) and side-chain (SC) types of POSS-kapton films.

FIGURE 1.54 The chemical structure of MC and SC POSS-Kapton films.
POSS-kapton films were exposed to AO in the space environment on the Materials International Space Station Experiment (MISSE) platform. The AO flux and fluence varied from experiment to experiment, depending on exposure duration, timing of the experiment within the solar cycle, and orientation of MISSE sample tray. The relative velocity of the AO with respect to ram surface is approximately 7.4 km·s−1, corresponding to oxygen atoms striking the surface with an average velocity of 4.5 eV. The MISSE-1 flight experiment was retrieved after 3.9 years. The samples were exposed to the ram and therefore all components of the LEO environment, including AO and VUV light, with the O-atom fluence being around 8×1021 atoms·cm−2, similar to the fluence on the companion MISSE-2 where the fluence was accurately measured to be 8.43×1021 atoms·cm−2.
The SC-POSS-kapton polyimide film was exposed to AO in the laboratory at room temperature, with a total fluence of about 2.7×1020 atoms·cm−2. Table 1.16 shows the laboratory AO erosion data of SC-Kapton film [92]. The experimental results indicate dramatic decreases in the surface erosion with increasing contents of SC POSS. Both SC POSS-Kapton and MC POSS-Kapton films exhibit comparable atomic-oxygen erosion yields. More specifically, in an atomic-oxygen exposure with a total fluence of 3.53 × 1020 O-atoms·cm−2, 7.0 %(w) Si8O12 SC POSS-Kapton film showed an erosion yield that was 3.3% that of Kapton H film. It was found that MC POSS-Kapton and SC POSS-Kapton films showed the comparable AO erosion yields for a given %(w) POSS cage.
TABLE1.16 Laboratory Atomic Oxygen Erosion Data of SC-Kapton Film

The effect of temperatures on the atomic-oxygen erosion yield of POSS-Kapton films with different Si8O12 loadings of 0%, 3.5%, and 7.0% were investigated. The samples were exposed to the hyperthermal O-atom beam at different temperatures of 25%, 100%, 150%, 220%, and 300 °C, respectively. Each sample set, corresponding to a particular temperature, was exposed to 50,000 pulses of the hyperthermal O-atom beam. Table 1.17 shows the laboratory AO erosion depths of MC-Kapton films at different temperatures. The erosion of the 0%(w) MC POSS-Kapton film control showed the strongest temperature dependence, with the erosion depth increasing by a factor of about 3.6 from 25 °C to 300 °C. And the 3.5 and 7.00%(w) Si8O12 MC POSS-Kapton films showed less temperature dependence in their erosion. The erosion depths of these samples increased by factors of 2.2 and 2.4, respectively, with the increase in temperature from 25 °C to 300 °C.
TABLE1.17 The Laboratory Atomic Oxygen Erosion Depths of MC-Kapton Films at Different Temperatures

The MC POSS-Kapton films with 0%(w), 1.75%(w), and 3.5%(w) were flown on MISSE-1 (ISS in 3.9 years). Various images of the samples were taken throughout the flight, and it was found that the 0%(w) MC POSS-Kapton film control sample was completely eroded in less than 4 months. On the basis of the estimated O-atom fluence of 8×1021 atoms·cm−2 and an erosion yield of 3.00×10−24 cm3 per atom for Kapton H film, the 0%(w) MC POSS-Kapton film should have eroded a minimum of 240 µm. Experimental results indicated that the 1.75%(w) and 3.5%(w) MC POSS-Kapton films were eroded by 5.8µm and 2.1 µm, respectively.
Miyazaki et al. investigated the tolerance of polysiloxane-block polyimide film against AO [93,94]. The commercially available silicon containing polyimide film (BSF30), a polysiloxane- block- polyimide is selected for investigation (Fig. 1.55). An AO beam was irradiated on the polysiloxane- block-polyimide film at the Combined Space Effects Test Facility of JAXA in Tsukuba, Japan. To investigate the AO tolerance, mass change measurement, cross-sectional transmission electron microscopic (TEM) observation, and X-ray photoelectron spectroscopic (XPS) analysis were performed.

FIGURE 1.55 Molecular structures of PMDA-ODA polyimide (SP-510) and Si-containing polyimide (BSF30).
Experimental results indicated that the mass loss of polysiloxane-block polyimide is only 1% or less than that of Kapton H. Cross-sectional TEM observation and XPS analysis revealed that the AO-protective SiO2 layer is self-organized by AO irradiation. Furthermore, the self-organized SiO2 layer is intentionally damaged to investigate reorganization of a new layer on it. AO irradiation of the damaged surface revealed that the new layer is built with a 500nm-deep eroded region. The “self-healing” ability of polysiloxane-block- polyimide was observed, suggesting that polysiloxane- block-polyimide film has high potential to provide many advantages of a space-use material, especially for LEO spacecraft.
A series of AO-resistant and transparent phosphine-containing polyimide coatings have been developed. A meta-substituted aromatic diamine, [3,5-bis(3-aminophenoxy) phenyl]diph- enylphosphine oxide (m-BADPO) was first synthesized by the Williamson reaction of 3,5- difluorophenyldiphenyl- phosphine oxide (DFPPO) and meta-aminophenol. The diamine was then polymerized with several commercially available aromatic dianhydrides to afford a series of aromatic polyimides (PI-1-PI-4) (Fig. 1.56). The meta-substituted molecular skeleton and the pendant bulky phenylphosphine oxide (PPO) group endowed the polyimides with desired properties for potential applications in space environments.

FIGURE 1.56 Preparation of transparent and atomic oxygen-resistant polyimides.
It was found that the solubility of the polyimides was enhanced due to the synergic effects of meta structure and the bulky PPO groups, making it possible to fabricate the polyimide films via solution procedure. The films exhibited flexible and tough natures with light color and high transparency in the visible light region (Fig. 1.57). Transmittance up to 87% at 400 nm was achieved in the films. PI-4 showed a cutoff wavelength at 319 nm, which was 7 nm lower than that of polyimide from p-BADPO and 6FDA (PI-4) at the same thickness. The transmittance of the PI-4 film at 400 nm (85%) was also superior to its para-linked analog (PI-4: 82%). Yellow index (YI) is usually adopted as a criterion evaluating the color of a polymer film. This value describes the color changes of a film sample from clear or white toward yellow. Lower YI value usually indicates a weak coloration for a polymer film. The YI values (b* values) of the m-BADPO-PI films (thickness: ~40 m) ranged from 6.4 to 13.4 dependent on the aromatic dianhydrides used. The YI values of 7.2 for PI-3 and 6.4 for PI-4 were apparently lower than those of their p-BADPO analogs (PI-3: 9.7 and PI-4: 8.0). The good transparency and low coloration of PI-3 and PI-4 films, on the one hand, could be attributed to its loose molecular packing induced by the bulky pendant PPO group in the diamine moiety and the flexible ether or 6F linkage in the aromatic dianhydride. On the other hand, the irregular structures caused by their meta-substituted molecular backbone were also advantageous to reducing the formation of charge transfer complexes (CTCs). Influence of the steric hindrance and inductive effects of the PPO groups and the meta structures on the optical properties of the polyimide films could also be reflected from the refractive index (n) values of the films. Generally, polymers containing substituents with low molar refractions or bulky molecular volumes often exhibit low n values according to the Lorentz-Lorenz equation. For instance, the Kapton type PI-(PMDA/ODA) has a n value of 1.6478 at 1320 nm, whereas PI-(6FDA/ODA) with a much looser molecular packing exhibits a lower n value of 1.5565. Obviously, the pendant bulky PPO substituents and the meta-substituted structure are expected to decrease the refractive indices of the polyimide films.

FIGURE 1.57 UV-visible spectra of the transparent and atomic oxygen-resistant polyimide films.
The polyimide films, PI-1, PI-3, PI-4 together with the Kapton standard (20×20× 0.05 mm) were exposed to AO at the ground-based simulation facility. The erosion rate of the Kapton was set to be 3.0×10−24 cm3·atom-1as a reference. The samples were exposed to AO with a total fluence of 8.13×1020 atoms·cm−2. Fig. 1.58 depicts the mass loss versus AO fluence for the polyimide films and Kapton reference. It can be observed that the polyimide samples containing the PPO group exhibited a weight loss of 5.48%(w)-8.76%(w) while that of Kapton H was 56.76%(w). In addition, the PPO-containing polyimide films exhibited a nonlinear weight loss rate while Kapton H exhibited a linear one. The erosion yields of the PPO-containing polyimide films were much lower than that of Kapton H. PI-3 shows the lowest erosion yield of 6.59×10−25 cm3·atom-1 while PI-4 exhibits the highest one. Obviously, the PPO groups in the present polyimides decrease their erosion yields in AO environments.

FIGURE 1.58 Mass loss versus atomic oxygen fluence for the transparent and atomic oxygen-resistant polyimide films.
The surface composition of the AO-exposed PI films was examined by XPS. The experimental results indicated that a layer of phosphorus oxide at the surface of PI-1 film was formed. This inert layer might inhibit the further erosion of the underlying polyimide film. Fig. 1.59 compares SEM images of Kapton H and PI-1 samples after AO exposure. Both of the surfaces of PI-1 and Kapton films exhibited a carpet-like appearance.

FIGURE 1.59 SEM images of Kapton H (A) and PI-1 (B) exposed to atomic oxygen (8.13×1020 atoms/cm).